By Tanmoy Chattopadhyay
X-ray astronomy unlocks a hidden universe of extreme events: from black holes and exploding stars to heated gas in galaxy clusters, it allows us to uncover the high-energy processes that have shaped the cosmos. As the next-generation X-ray missions (for example, the Lynx Observatory) probe ever deeper into the faint, high-redshift Universe in search of the first stars and galaxies, the current generation of detectors will need upgrades to support their capabilities. For example, the new observatories will capture more photons with their larger mirrors, and as input increases, so does the potential for electronic noise that will lower the quality of the data.
The hardware wing of the X-ray Astronomy and Observational Cosmology (XOC) group led by KIPAC professor Steve Allen, in collaboration with MIT and MIT’s Lincoln Laboratory (MIT-LL), is developing a novel technology called Single-electron Sensitive Read Out (SiSeRO). SiSeRO detectors could provide an order-of-magnitude improvement in noise and speed performance over the charge-coupled devices (CCDs) used in major X-ray telescopes like the Chandra X-ray Observatory and XMM-Newton. Our progress was published in the Journal of Astronomical Telescopes, Instruments, and Systems (January 2024).
SiSeROs or CCDs?
CCDs are the true workhorses of the digital imaging world and have been used in many space-based telescopes, capturing light from visible to lower-energy (soft) X-rays. In simple terms, CCDs work by converting incoming photons to electric charge (Fig. 1). When multiple CCDs (also called pixels) are arranged in a grid, these “charge packets” can be read out and interpreted as an image.
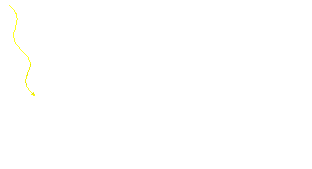
The basic principle behind SiSeROs is the same as for CCDs. However, CCDs do something SiSeROs do not: they destroy the charge packet during the readout process (not shown in Fig. 1). SiSeROs, on the other hand, move the charge to a vacant channel under a MOSFET (metal-oxide-semiconductor field effect transistor) at the output, changing the current in the transistor, where the change in the current is proportional to the charge. By reading out this change in current with the charge kept intact in the vacant channel, we perform an indirect measurement of the charge without destroying it. Since the charge remains unaffected, a key advantage is that we can potentially measure it multiple times and average out the electronic noise. This is called repetitive non-destructive readout (RNDR).
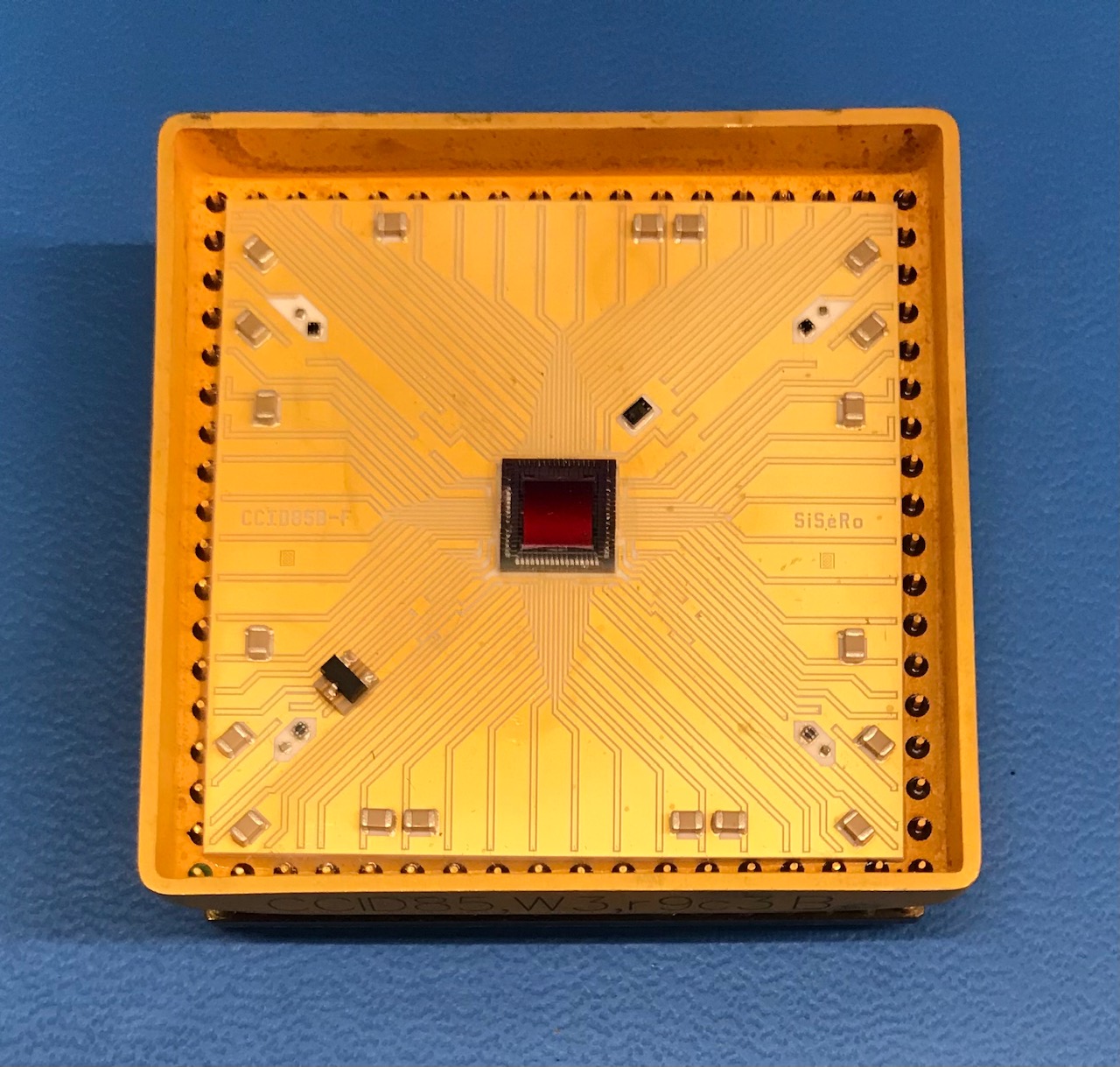
Such low noise (less than that caused by one electron) opens opportunities for calibration of the instrument with unprecedented precision. Fig. 3 shows a simulated distribution of the number of electrons resulting from an input photon energy of 100 electron-volts (eV, in red). While most of the time, the deposition of 100 eV generates 27 electrons in the silicon (3.65 eV is needed to generate one electron in silicon; 100 eV / 3.65 eV = 27 electrons), there are some instances where it can generate more or fewer than 27 electrons. When the read noise is ⅓ of an electron or lower, we can begin to resolve individual electron bumps in the distribution and measure the total deposited energy with high accuracy. For comparison, the black line shows the same 100 eV line spectrum for a CCD-like detector with two electrons of noise, which smothers the signals from the individual electrons.
With such high precision and low noise, where even single electrons can be detected, the detectors are able to measure much lower-energy photons in the soft X-ray regime. This enables accurate measurement of such phenomena as the circumgalactic medium (CGM) that fills the dark matter halos of galaxies, stars and their impact on exoplanet atmospheres, and the deepest sky surveys, where cosmic expansion causes the emission spectra of the first galaxy groups and clusters and the earliest active galactic nuclei to shift down to soft X-ray energies.

Proof of Concept demonstration
We started working on these detectors in 2019. Recently, the first-generation SiSeRO prototypes have shown some encouraging results. The prototypes are based on established CCD technology with a primary goal of demonstrating a working SiSeRO output stage.
Even though the prototypes are not yet optimized for RNDR measurements (the dedicated parking gate necessary for storing the charge has not yet been implemented), we were still able to test their performance in noise reduction. Figure 4 (right) shows the first results of this proof-of-concept experiment, where the readout noise decreases as the number of RNDR cycles increases. We were able to reduce the noise by a factor of 7.25 after averaging over 57 RNDR cycles.

While RNDR achieves low noise by measuring the same charge multiple times, it slows down the readout speed. To compensate, we plan to build a SiSeRO detector where each pixel has its own amplifier to measure the charge signal. SiSeRO readout in each pixel will boost readout speed significantly, allowing the study of fast-changing X-ray sources like pulsars. The faster readout also reduces particle background noise. A prototype is being fabricated at MIT-LL, and we plan to build a larger SiSeRO detector. Such technology will open a path towards fast, low-noise megapixel spectral imaging for future X-ray observatories.
Edited by Lori White, Toby Satterthwaite, Xinnan Du, Josephine Wong, Sanskriti Das, and Jack Dinsmore
